![]() |
|
||||
BiographyJosef Weinbub is an Assistant Professor (tenure-track) of High Performance Simulation in Micro- and Nanoelectronics and an IEEE Senior Member. He obtained the doctoral degree in Computational Microelectronics and the venia docendi (habilitation) in the field of Micro- and Nanoelectronics from the TU Wien. He was a visiting researcher at the EPCC, University of Edinburgh and at the Device Modelling Group, University of Glasgow, Scotland, UK as well as at SILVACO Inc., Santa Clara, CA, USA. He founded and now chairs the master’s program Computational Science and Engineering at the TU Wien and is involved with several international scientific conferences in various management and scientific roles. He is an Associate Editor of the Journal of Computational Electronics and a Principal Investigator of various research projects funded by, e.g., the Austrian Science Fund and the Christian Doppler Research Association. Together with his team he investigates cutting-edge research problems in the area of computational micro- and nanoelectronics. |
Modeling Coulomb Interaction with a Wigner-Poisson Coupling Scheme
Entangled quantum particles, for which operating on one particle instantaneously influences the state of the associated particle, are attractive for carrying quantum information at the nanoscale. However, describing entanglement in traditional time-dependent quantum transport simulations requires doubling the degrees of freedom, involving an almost prohibitive computational effort. Considering electrons, one approach to analyzing their entanglement is by modeling the Coulomb interaction (Coulomb Entangler) via the Wigner formalism.
We showed that the computational complexity of the time evolution of two interacting electrons is reduced by approximating the Wigner equation in the two-particle phase space using two equations, each defined in a single-particle phase space. In particular, we replace the non-local Wigner potential operator of the electron-electron interaction with an electrostatic field that is introduced through the spectral decomposition of the potential. The coupling between the two equations, based on a consecutive update of the electric field, establishes the entanglement. It can be demonstrated that for certain particular configurations of an electron-electron system, the introduced approximations are feasible. Purity, identified as the maximal coherence for a quantum state, is analyzed, and it is consequently demonstrated that entanglement due to Coulomb interaction is well accounted for by the introduced local approximation.
Fig. 1 (left) shows the purity of two electrons without Coulomb interaction. The first electron is injected into the simulation region within 60 fs. After the complete injection of the electron, the uncertainty rule is satisfied, and the purity stabilizes at the perfect value one, which prevails until the electron starts to exit the simulation region after about 190 fs. The injection of the second electron begins 70 fs after the first one, indicated by the blue curve. In the time interval between t = 140 fs and t = 190 fs, highlighted by the dashed red lines, both electrons evolve quantum mechanically, without interaction, as two independent pure states. Fig. 1 (right) focuses on this time interval and shows the purity being perfectly equal to one. Fig. 2 (left) demonstrates the effect of Coulomb repulsion. To better highlight the influence, the charge of each electron is increased by an order of magnitude. Accordingly, at t = 140 fs, the purity starts to drop at the beginning of the interaction. The level of entanglement due to Coulomb interaction increases during the evolution, which is well demonstrated by the continuous decline in the purity. As can be seen in Fig. 2 (right), the purity evolves equally for both electrons as expected.
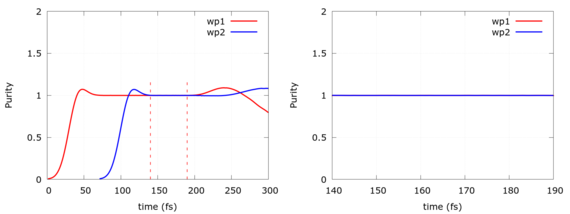
Fig. 1: Time evolution of the purity of two non-interacting wavepackets (i.e. electrons) for (left) the entire evolution time (dashed red lines indicate time interval shown right) and (right) the time interval from t = 140 fs to t = 190 fs. Reprinted with permission from Benam et al., J. Comput. Electron. (2021). Copyright 2021 Author(s), licensed under Creative Commons Attribution 4.0 International.
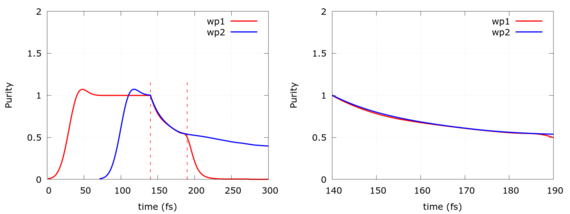
Fig. 2: Time evolution of the purity of the two states in the Coulomb Entangler for (left) the entire evolution time (dashed red lines indicate time interval shown right) and (right) the time interval from t = 140 fs to t = 190 fs. Reprinted with permission from Benam et al., J. Comput. Electron. (2021). Copyright 2021 Author(s), licensed under Creative Commons Attribution 4.0 International.